#
End-Use of Low-Carbon Resources
# Introduction
Ultimately, customer preferences and costs will drive investment and adoption decisions that will define, in aggregate, the trajectory of economy-wide decarbonization. Therefore, assessing the viability of low-carbon pathways must include an analysis of decision-making processes by the end-users of energy regarding their energy requirements. This analysis should be built upon an understanding of the range of end-use devices, equipment, and processes in each market sector. Energy conversion efficiency, performance and operational impacts, comfort and convenience at the point of use, product quality impacts, health/safety/environmental risks, upfront capital cost, technology maturity, and stranded asset risk are just some of the factors that must be considered in addition to energy cost in order to properly characterize the relative competitiveness and adoption potential of prospective low-carbon pathways. Due to the complexity and interaction of these factors, decarbonization pathways will vary significantly between market sectors, regionally within sectors, and between market sub-sectors.
With this in mind, the LCRI End-Use TSC has approached the analysis of the landscape of AEC-based decarbonization pathways and technologies from a customer segmentation and final energy application perspective. Work is divided into three major end-use sectors: Buildings, Transportation, and Industry. Figure 24 provides a breakdown of the specific applications and market segments currently being considered within each of these sectors. Detailed landscape assessments of decarbonization pathways for each of these market segment and sub-segments have been developed and used to inform this roadmap.
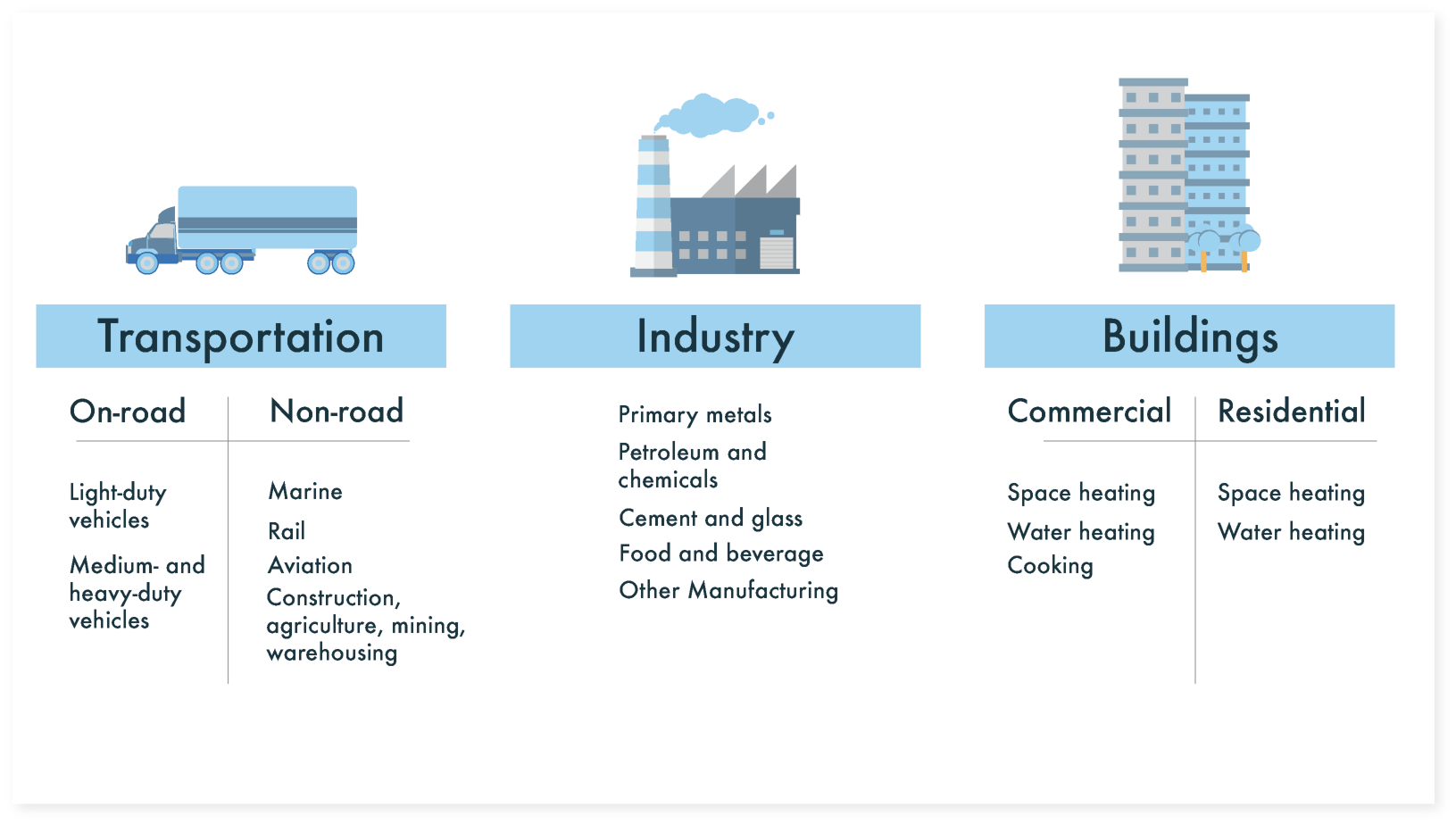
In each case the most significant end uses of fossil fuels (that is, those with the largest carbon footprint) in each sector were identified and prioritized by this TSC based on:
The current status of key low-carbon technologies and key barriers to their application;
The relative market position of AEC pathways to other competitive decarbonization options (for example, direct electrification);
Key RD&D needs that must be addressed to enable and/or accelerate adoption of low-carbon technologies, informed by input from advisors and key stakeholders.
The following sections provide a more detailed characterization of competing decarbonization pathways and research needs for AEC-based approaches for the Buildings, Transportation, and Industry sectors.
# Transportation
LCRI research interest in transportation encompasses the wide variety of methods to move or carry goods and people. Transportation is the highest-emitting sector of the U.S. economy and represented ~17% of GHG emissions globally in 2018 (WRI 2021). Today, the transportation sector is almost entirely dependent on petroleum fuels, although there is a notable use of biofuels and a small, but growing, use of electricity. While continued efficiency improvements and electrification of certain modes of transport are expected to contribute to emissions reductions, fuel switching to AECs will likely play a critical role in enabling deep carbon reductions across the transport sector.
Looking forward, electrification appears to be a very promising decarbonization option for the majority of light-duty vehicles. Most medium- and heavy-duty on-road vehicles are likely to be technically electrifiable, but barriers to electrification exist for transportation market segments that require longer ranges or heavier payloads due to the relatively low energy density of batteries. The weight, volume, and fueling times of AECs offers advantages for these transportation needs. Specific applications that fall into this class include:
Marine shipping;
Aviation;
Long-distance trucks and buses with high load intensity; and
Rail, particularly for long-distance routes with lower network utilization (for example, rail freight).
Certain heavy-duty construction, agricultural, and mining equipment.
Currently, there is significant global effort surrounding hydrogen fuel cell electric powertrains for medium- and heavy-duty, on-road vehicles, with several vehicle manufacturers developing commercial offerings. Over a thousand fuel cell electric trucks and delivery vehicles are currently operational in China alone, and thousands of fuel cell electric buses are set to roll out by 2025 (IEA 2019b). Drop-in fuels that replace the use of fossil-sourced liquified natural gas with RNG or SNG or replace conventional diesel with biodiesel are also potential decarbonization solutions for this market segment.
In the marine space, methanol and biofuels such as hydrotreated vegetable oil/biodiesel are in early commercial deployment, with ammonia- and hydrogen-fueled marine propulsion systems at earlier stages of development. For the rail segment, a small number of hydrogen fuel cell trains currently operate in Europe, and several countries have announced plans to develop and deploy hydrogen-fueled rail. Biofuels and synthetic fuels are potential diesel substitutes for existing non-electric rail routes, but experience with use of these fuels in the rail sector has been limited to-date. There is also notable momentum in research and demonstration of AEC-fueled heavy-duty vehicles used in the construction, agricultural, and mining sectors.
# Key Research Questions
Over the course of the LCRI, the TSC intends to address the following research questions:
What is the relative technical and economic feasibility of competing decarbonization pathways across the transportation sector? In which applications are AECs expected to be the most competitive and impactful at driving emissions reductions?
How will adoption of AECs impact performance and total ownership costs among key on-road and non-road vehicle classifications across the transportation sector?
How, and to what degree, can existing engine and on-board fuel supply and storage system technology and fueling infrastructure be retrofitted to accommodate non-drop in AECs?
Which AECs will be embraced by manufacturers and end users in the transport sector? What are the training, workforce development, and customer education needs to enable their widespread adoption?
What are the costs and challenges for end-point fueling infrastructure installation and operation? What are the maintenance, inspection, and other operational impacts relative to incumbent fueling operations?
How can fueling stations be deployed to provide sufficient coverage for targeted usage patterns and alternative fuels?
# Research Goals
A summary of the proposed research effort and associated goals is provided below.
# Goal 1. Understand the Costs and Technical Characteristics of AEC-Fueled Vehicles
Strategy 1: Evaluate costs of AEC-fueled vehicles in key on-road and non-road applications.
Strategy 2: Analyze how transition to AECs impacts vehicle performance, including efficiency, mass/volume of powertrain, range, refueling time, acceleration, and emissions.
Strategy 3: Assess the EHS and vehicle maintenance impacts of low-carbon fuel adoption.
Strategy 4: Evaluate the technoeconomic feasibility, relative competitiveness, and GHG abatement potential of alternative-fueled vehicles compared to electrification and fossil fuel base cases.
# Goal 2. Understand the Technical Characteristics and Deployment Trajectory of AEC Fueling Stations
Strategy 1: Assess endpoint fueling infrastructure installation and operational costs for key on-road and non-road applications.
Strategy 2: Perform modeling to characterize deployment scenarios for AEC fueling infrastructure to achieve sufficient coverage for targeted usage patterns and fuels and understand the value of fueling station design and operational flexibility.
# Goal 3. Identify, Evaluate, and Advance High-Priority Technologies
Strategy 1: Prioritize AEC applications on a basis of technoeconomic feasibility, competitive position, and GHG abatement potential.
Strategy 2: Evaluate and monitor ongoing RD&D efforts for prioritized AEC-based pathways and address critical research gaps through development and demonstration efforts.
# Joint Areas of Research with Other Technical Subcommittees
The list below outlines areas where joint research with, and integration of, activities from other TSCs will be necessary.
Collaboration with the AEC production TSCs will inform supply considerations such as projected costs and availability.
The Delivery and Storage TSC will provide key input on AEC distribution and storage infrastructure and safe handling practices, particularly as these topics relate to refueling sites/stations.
# Industry
Globally, 79% of industrial final energy is derived from fossil fuels, 56% of which is used for direct process energy inputs while the remaining 44% is feedstock for the production of other chemicals or intermediates (McKinsey 2020). In 2016, industrial activity resulted in 17,078 MMt CO2e or 29.4% of annual global GHG emissions, of which 2,567 MMt CO2e was liberated by in-process chemical reactions (WRI 2021).
# Process Heating Applications
Currently, fossil fuels are widely used in industrial applications to supply process heat. In the U.S., industrial activity accounts for 20% of the economy-wide energy consumption and over a quarter of all combustion related CO2 emissions. Direct fossil fuel process heating and steam boiler technologies are deeply embedded in these processes, constituting 57% of all industrial final energy use (EIA 2021). Combined heat and power and advanced heat recovery solutions are solutions that could initially be deployed as transitional technologies that reduce fossil energy consumption. In addition, in certain cases these processes could be cost-effectively decarbonized through electric technologies such as resistance, infrared, induction, and radio frequency heating, microwave and ultraviolet initiated processing, and process intensification (EPRI 2021b).
AECs may also provide competitive solutions for decarbonizing industrial process heat applications. Examples applications where they might be competitively applied include those requiring high volumes of high-pressure steam, such as paper drying, natural gas reforming for petroleum product hydrotreating, chemical process thermal inputs, food and beverage cooking, sterilization and pasteurization, and steel mill power generation.
# Feedstock Applications
AECs are currently used as feedstocks or being explored as substitute feedstocks for a variety of industrial sectors. For example, the oil refining and chemical production sectors dominate current demand for hydrogen, which is supplied by fossil fuel-based, carbon-intensive production routes like SMR or coal gasification. This hydrogen supply could be decarbonized by substituting unabated, fossil-derived hydrogen with low-carbon hydrogen generated by electrolysis powered by clean energy or SMR with CCS. In addition, new industrial feedstock applications of AECs are emerging to replace existing carbon-intensive inputs. For example, an emerging steelmaking process enables replacement of coking coal-derived reducing gases with hydrogen as a reducing agent.
# Process-Related Carbon Dioxide Emissions
In some industrial sectors a significant proportion of CO2 emissions result from liberation during process related chemical reactions. For example, global process emissions from the production of clinker in the cement industry results in 1500 MMt CO2 annually, or 3% of total global carbon emissions (Hasanbeigi 2019). Similarly, the reduction of iron results in the liberation of 1100 MMt CO2 annually, or 2.2% of global emissions (worldsteel 2020a)[1]. Similar process-related CO2 emissions occur in a variety of petroleum and chemical industry processes. In certain cases, a highly concentrated CO2 stream may make a CCS solution viable, including capture of any other site emissions such as those from combustion. Additionally, the use of the captured CO2 in a variety of end products in these industries should be evaluated as a circular economy approach to support decarbonization. For example, certain Portland cement chemistries have been developed that utilize CO2 in the curing process.
# Key Research Questions
Over the course of the LCRI, the End-Use TSC intends to address the following research questions.
Which AECs will be readily accepted and embraced by industrial processes due to the relative ease of transition (that is, "drop-in" compatibility), including consideration of health, safety, and environmental consequences?
What are the application-specific technoeconomic barriers to use of AECs for process heating or as chemical feedstocks? What is the technoeconomic potential for hydrogen, hydrogen/natural gas blends, and other AEC pathways to achieve competitiveness with a variety of direct electrification technologies for industrial process heat supply?
What equipment modifications are required to replace fossil fuels with low-carbon alternatives in steam boilers, direct combustion furnaces and heaters, gas turbine compressor drives, and combined heat and power systems?
What are the potential environmental concerns resulting from the production and combustion of hydrogen, ammonia, or other low-carbon alternative fuels for industrial applications, such as increased nitrous oxides (NOx) emissions? What is the relative effectiveness of potential mitigation technologies such as CCS, catalytic abatement, or scrubbing?
What is the regional demand for AECs based on current and future projected geographic concentrations of specific industrial subsectors and considering demand competition with other end uses?
What is the technoeconomic potential for industrial applications of carbon capture and carbon utilization in industrial processes, such as synfuel production and sequestration of captured process CO2 in industrial materials such as concrete?
What is the technoeconomic potential of alternative production processes that eliminate process CO2 emissions, such as iron ore electrolysis and direct hydrogen reduction in the steelmaking sector or supplemental cementitious materials?
What are the equipment costs and energy performance (that is, effectiveness and efficiency) of thermal energy use from alternative AECs in industrial combustion systems for steam and direct process heating, combined heat and power, and machine drives?
# Research Goals
A summary of the proposed research effort and associated goals is provided below.
# Goal 1: Identify Competitive Decarbonization Pathways
- Strategy 1: Develop analysis of decarbonization pathways that are integrated across the entire fuel and CO2 supply/disposal chain to deliver targeted fuel costs at industrial sites
# Goal 2: Conduct Impact Assessments
Strategy 1: Assess the impact of switching from conventional fuels to AECs on existing industrial end uses (for example, boilers, furnaces, heaters, ovens, turbines, and engines)
Action: Identify and assess impacts on materials, equipment performance, and operations.
Action: Examine a wide range of AECs and blends compared to incumbent fuels and electric alternatives.
# Goal 3: Develop Technoeconomic Assessments for Equipment Retrofits and New Equipment
Strategy 1: Investigate the safe and cost-effective reuse of existing assets with re-engineered combustion fuel trains and burner configurations
Action: Identify cost-effective retrofit materials, equipment, and technologies needed to extend the life of existing assets with use of AECs.
Action: Evaluate the technical and economic feasibility of deploying retrofit technologies.
Strategy 2: Investigate the safe and cost-effective development of new technologies designed to use AECs
Action: Evaluate new materials, equipment, and technologies needed to replace existing assets, expand capacity, or develop a completely new process technologies compatible with AECs.
Action: Evaluate the technical and economic feasibility of new AEC compliant assets.
# Goal 4: Identify and lead efforts to accelerate advanced technology development
Strategy 1: Identify and evaluate emerging technologies that could lead to cost-competitive applications of AECs
Action: Monitor emerging technologies to identify innovative materials and processes.
Action: Prioritize potential bench or small-scale demos to evaluate feasibility and accelerate adoption as technologies emerge.
# Joint Areas of Research with Other Technical Subcommittees
The list below outlines areas where joint research with, and integration of, activities from other TSCs will be necessary.
Collaboration with the Electrolytic Processes, Hydrocarbon-Based Processes, and Delivery & Storage TSCs will be necessary to understand specific physical attributes of the delivered AECs that are critical to end users such as purity, temperature and pressure requirements, and physical phase.
Input on the costs and logistics of fuel production, storage, and delivery will be required to conduct technoeconomic analysis of the cost competitiveness of each of the AEC pathways and to determine the most beneficial end uses on an economy-wide basis as a means of prioritizing future research.
Joint efforts with the Power Generation TSC will focus on industrial power generation equipment (for example, boilers, reciprocating engines, gas turbines) considerations such as material and process compatibility and reliability.
# Buildings - Commercial & Residential
The buildings sector accounts for 28% of global energy-related CO2 emissions—an increase of more than 25% since 2000—and 30% of global final energy use. Space conditioning (that is, heating and cooling), water heating, and cooking account for more than 75% of energy demand in buildings, making these applications the major focus for GHG emissions reductions in this sector (IEA 2019d).
The ongoing decarbonization of the electric power grid creates opportunities for electrification technologies to contribute to a low-carbon future in both new and retrofit buildings. Efficiency improvements—driven by advancements in lighting and appliances, insulation and space conditioning technologies, and other advanced conservation measures—buildings-integrated renewables and energy storage solutions, deep energy retrofits, and digitalization/advanced demand-side response tools are also expected to contribute to the reduction of buildings' GHG footprints while mitigating seasonal load peaks. The highly distributed, fixed locations and predicable demand of energy end uses in the buildings sector enable competitive fixed distribution networks for both natural gas and electricity in space and water heating, cooling systems, and cooking applications.
With more than half of U.S. homes and businesses served by delivered fuels (U.S. Census Bureau 2021), supplementing or substituting these existing natural gas, propane, and fuel oil loads with low-carbon alternatives is critical to achieving decarbonization goals. The direct application of AECs in residential and commercial buildings is being explored to reduce the carbon intensity of existing natural gas end uses and also displace higher-carbon liquid and solid fuel systems. These alternative fuel-based pathways could play an important role in decarbonizing building applications where space conditioning and water heating are more difficult or costly to electrify, such as in regions with older building stocks or sharp winter heating load peaks (EPRI 2020). "Drop in" compatible biomethane/RNG or SNG could be injected into natural gas pipelines to reduce the carbon intensity of natural gas end uses.
Existing natural gas networks could be utilized to deliver hydrogen which, when blended at relatively low levels (up to around 20% by volume), could be safely utilized by existing gas-fired equipment in residential and commercial buildings (AHRI 2021). However, delivering 20% hydrogen by volume blended with natural gas would only reduce CO2 emissions by approximately 7% due to the lower volumetric energy density of hydrogen. Meanwhile, supply of pure hydrogen via existing gas networks would require extensive infrastructure and end-use appliance modification or replacement. Depending upon regional characteristics, these end use requirements can be the most restrictive conditions on hydrogen blending tolerance in natural gas networks (NREL 2013), and more work is needed to understand the long-term impact of blended hydrogen on building gas distribution systems and gas-fired appliances. Supply of pure hydrogen is being explored as a pathway to decarbonize gas networks in the longer term, but this approach would require extensive distribution infrastructure and demand-side equipment modification or replacement (EPRI 2020). In Europe, some end use equipment is currently being developed for operation on pure hydrogen, but more work is needed to fill out a product portfolio of hydrogen-fired equipment.
As end-use load flexibility and energy system resilience are prioritized by utilities and end users, assuring redundancy in the built environment is increasingly important. Thus, developing and deploying cost-effective approaches to combined heat and power is critical, assuring that these technologies (for example, stationary engines, turbines, and fuel cells) are designed, installed, and operated to support building decarbonization goals. Similarly, on-site energy storage technologies can be optimized to support resilience goals while mitigating peak energy demands. These solutions represent opportunities for AEC utilization in buildings and can be deployed at both the individual building and community scales. Distributed carbon capture solutions are also emerging that can capture CO2 directly from combustion equipment in large buildings, which may provide transitional emissions abatement strategies for difficult-to-decarbonize buildings, particularly in urban centers where economies of scale could arise for CO2 collection, distribution, and utilization infrastructure.
Due to the extraordinary diversity of the global building stock, with variation in end use equipment, construction and design techniques, age and remaining lifespan, embedded/available infrastructures, climate zones, building codes and standards, and socioeconomic strata, a broad portfolio of solutions will be required to meet the challenge of buildings decarbonization. An additional complication is the long lives of buildings—for example, the average lifespan of a U.S. home is 130 years (Ianchenko 2020)—which means the majority of the effort in decarbonizing buildings within the 2050 timeframe concerns buildings that exist today. Thus, different decarbonization solutions will be applied in existing buildings served directly by fuels (for example, natural gas, propane, and fuel oil) compared to new buildings whose energy needs may mostly be served by electricity. As such, this TSC describes two primary "branches" of decarbonization pathways: a primarily fuel-fired pathway and a primarily electric pathway, wherein applicable buildings and technology needs will differ approximately per Figure 25. While the latter pathway is expected to play a significant role in building decarbonization, the research focus for LCRI will be on fuel-fired pathways.
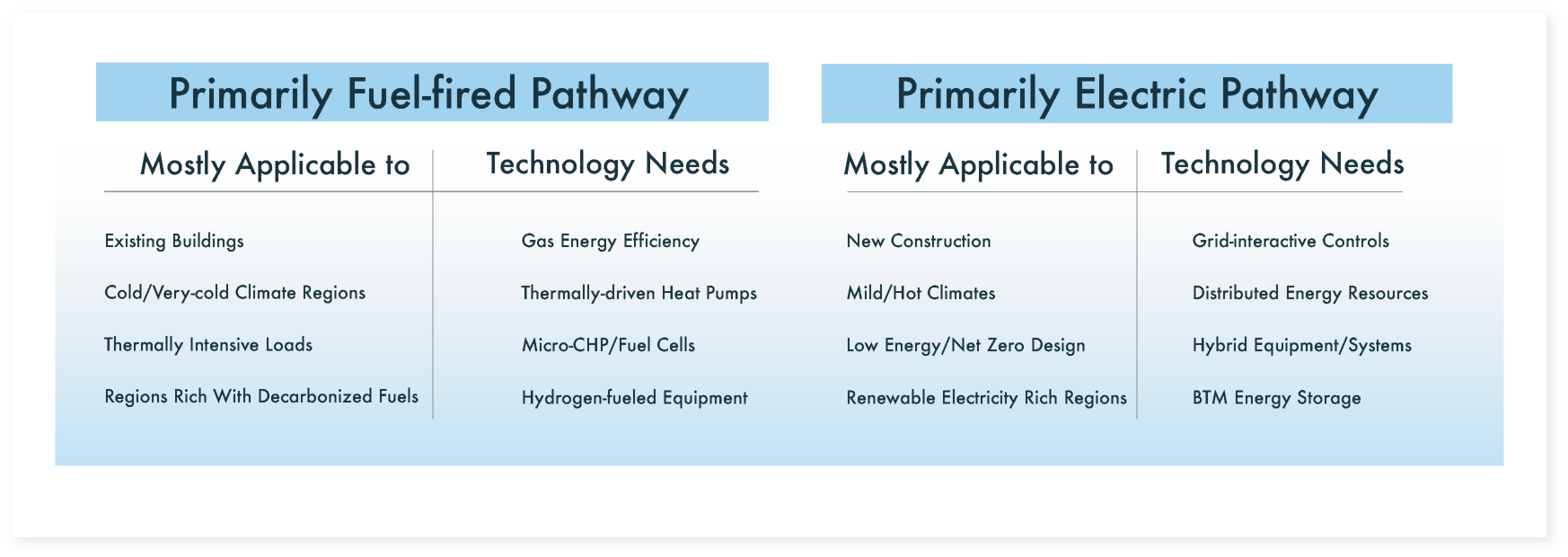
# Key Research Questions
Over the course of the LCRI, the TSC intends to address the following research questions.
What is the technoeconomic potential of use of both AEC/natural gas blends and 100% AECs in buildings for space conditioning, water heating, cooking, and other end uses; and what is the competitiveness of these approaches with alternative building decarbonization pathways (for example, electrification, hybrid dual-fuel equipment)?
What impact would variable natural gas-hydrogen concentrations have on demand-side equipment and appliances? What impact would various natural gas-hydrogen compositions have on the equipment, flame, and exhaust of existing natural gas appliances?
What customer education/acceptance and installer training needs exist to enable adoption of AECs in residential and commercial buildings?
What RD&D needs exist to advance direct carbon capture technologies for buildings? In which applications is it positioned to become a cost-effective decarbonization option?
What retrofits/after-market modifications to existing end use equipment in buildings is needed to enable utilization of AECs? What retrofit or new construction approaches are required to safely distribute, store, and utilize hydrogen within building footprints?
What role could on-site storage and utilization of AECs play in ensuring resiliency and effective/scalable approaches to provision of demand-side grid services?
What steps need to be taken to develop systemic, scalable, and equitable approaches to decarbonization of existing homes via AEC pathways? What are the cost, health, safety, and environmental implications of building decarbonization measures on low-income and marginalized communities?
What new maintenance practices, codes and standards, and technologies (for example, leakage detectors) need to be developed to ensure safe and reliable usage of hydrogen in homes and businesses?
# Research Goals
A summary of the proposed research effort and associated goals is provided below.
# Goal 1: Develop an Analytical Framework to Identify Decarbonization Pathways for Building Sub-Sectors
Strategy 1: Develop a set of drivers, including local characteristics, to consider in analyzing scenarios for building stock transformation over time
Action: Identify a set of applicable options and tradeoffs (for example, beneficial electrification, direct use of AECs, hybridized approach).
Action: Identify regional concerns, current and projected business-as-usual energy loads and demands, and other features.
Strategy 2: Utilize a combination of established and custom analytical tools to frame the breadth of building decarbonization options
# Goal 2: Conduct Technoeconomic Assessment of Viable AEC-Based Decarbonization Pathways
Strategy 1: Develop boundary conditions and assumptions to complete initial assessment
Action: Determine the approximate cost of delivered AECs, comparative equipment upgrade/conversion costs, the importance and quantification of resilient energy systems/'outlier' events (for example, extreme weather), and other critical considerations.
Action: Quantify and compare the energy, emission, and cost impacts across the segmented building stock in a 2030 and 2050 scenario.
# Goal 3: Identify and lead efforts to accelerate advanced technology development
Strategy 1: Identify and evaluate emerging technologies that could lead to cost-competitive applications of AECs
Action: Monitor emerging technologies to identify innovative materials and processes.
Action: As technologies emerge, prioritize potential bench or small-scale demos to evaluate feasibility and accelerate adoption.
# Joint Areas of Research with Other Technical Subcommittees
The list below outlines areas where joint research with, and integration of, activities from other TSCs will be necessary.
The Storage and Delivery TSC will provide necessary insight into the safe distribution, metering, and on premises storage of AECs within the property boundary of one or a collection of buildings.
Building- and community-scale power generation will necessarily require the expertise of the Power Generation TSC to enable deeper understanding of the technical considerations of converting AECs to electricity and/or heat at or near the point of use.
For further discussion of potential routes to decarbonize steel or concrete, see Appendix B. ↩︎